1. Why aren’t solar PV and wind power enough?
Solar PV and wind power have accelerated their development dramatically in recent years. In 2023 alone, over 510 GW of new renewable generation capacity was connected to power grids worldwide. In Europe, renewable electricity covered no less than 44% of electricity consumption in 2023. According to the IEA, the outlook for PV and wind power worldwide is now in line with the Net Zero 2050 scenario. Which is excellent news for the Energy Transition!
But there is a “BUT”. It would be a mistake to believe that these two renewable energies are the Alpha and Omega of the Energy Transition. After all, they both have three disadvantages that need to be offset if we are to meet the objectives of the Paris Agreement:
- They are intermittent energies, available only when there is sun or wind, not always when there is a high demand for electricity.
- Electricity is not easily stored, and batteries will only be able to cover part of the storage needs.
- Finally, the massive development of solar and wind power poses problems for grid stability.
The first disadvantage can be partly offset by the development of another source of low-carbon electricity: nuclear power. But Europe’s nuclear power plants (notably in France) are old and currently insufficient to cover future needs for decarbonized electricity. And the construction of new nuclear power plants will take time: with a lead time of around 15 years between the decision to build and the commissioning of the plant, these new non-intermittent decarbonized energy production capacities will not arrive before 2035-2040. Only China, with its faster construction times, should be able to move ahead more quickly.
The second problem is the difficulty of storing electricity to compensate for the intermittent nature of wind power and PV. The two mature technologies that make this possible are hydraulic pumped-storage stations (STEPs) and batteries. STEPs are a proven and effective solution, but the vast majority of sites in Europe are already equipped. And current battery technologies can only store a few hours’ production from large-scale PV or wind power plants (the largest grid battery projects currently in operation store little more than two or three hours’ production).
Finally, the problem of power grid instability in the presence of more than 50% PV or wind generation stems from a limitation linked to the physics of large-scale power grids. To be stable, they need to be powered by high-power rotating machines (the alternators of large thermal power plants), which have a high degree of rotational inertia. In the event of a power demand peak, the inertia of these large alternators prevents the machine from slowing down, and absorbs the peak without any problem, without any drop in rotation speed, and therefore frequency. On the other hand, when there are a majority of PV panels or wind turbines, these do not provide the beneficial moment of inertia of large thermal power plants. Stability must then be artificially created by adding batteries. That is why it is essential to install batteries on the grid at the same time as PV and wind power, to ensure the necessary stability by storing and releasing energy for short periods. As mentioned above, these same batteries can also be used to store surplus renewable electricity for a few hours.
This is exactly what the market has understood! The latest IEA study on stationary batteries (“Batteries and Secure Energy Transition”, IEA, 2024) shows just how fast grid batteries are currently developing (see figure below).
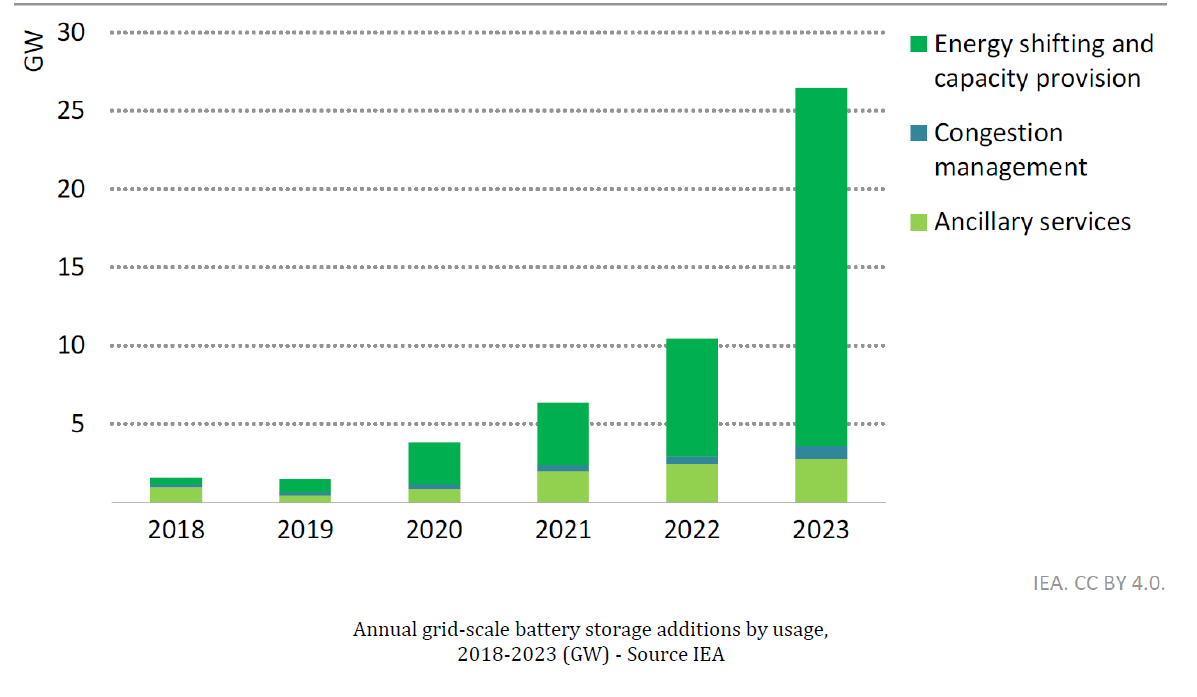
The pace of development is set to increase even further: in the Net Zero (NZE) scenario, it is set to increase 35-fold between 2022 and 2030, to reach almost 970 GW! Around 170 GW of new capacity will be added in 2030 alone, compared with 11 GW in 2022 and 26 GW in 2023. And of course, such exponential growth poses the problem of the availability of raw material resources, particularly lithium.
To sum up, the decline in fossil fuels today relies to a large extent on the development of wind and photovoltaic electricity. This calls for massive investment in battery storage capacity on electricity grids, with the risk of a bottleneck in the supply of the necessary raw materials, notably lithium. To diversify renewable energy sources, we urgently need to consider other possible solutions, notably non-intermittent renewable energies and thermal storage.
2.Biogas and biomethane
Biogas and biomethane are among the non-intermittent renewable energies of particular interest for the Energy Transition, as they enable all uses of natural gas to be decarbonized, including those for which it is difficult or impossible to switch to electricity (certain industrial thermal processes, heavy transport, etc.), without increasing demand for renewable electricity.
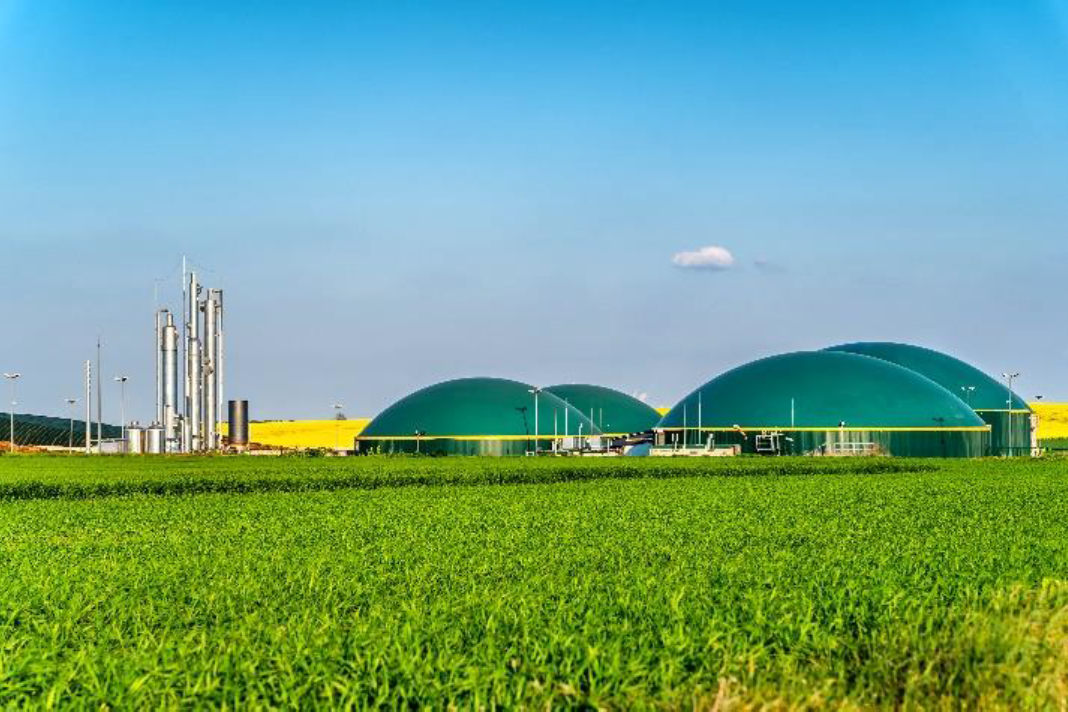
2.1.Methanization
At the same time, the race for size continues. Equinor has announced the commissioning of an 88 MW floating wind farm in Scotland. Scheduled to open in 2023, this will be the world’s largest floating wind farm. And manufacturer Vestas, which has been working on a new 15 MW offshore wind turbine with a production capacity 50% higher than that of the previous generation, has just signed its first installation in a floating offshore wind farm in South Korea in 2023.
Today, biomethane is mainly produced by the methanization of wet waste: agricultural residues, waste from the agri-food industry (dairy industry, etc.), household waste, sewage plant sludge, etc. After pre-conditioning, the inputs are fed into a methanizer, where the biological fermentation reaction takes place, generating a methane-rich gas: biogas. This gas is then purified to transform it into methane that meets the purity specifications imposed by gas network operators, enabling it to be injected into the networks: this is known as biomethane. At the same time, the methanization process produces a residue containing all non-methanizable inert matter: digestate. Digestate is generally used as a natural fertilizer, reducing the need for synthetic fertilizers produced from fossil fuels, and providing additional income on top of the proceeds from the sale of biomethane.
Biomethane produced in this way can be used as a substitute for fossil natural gas in all applications: fossil-fired processes, natural gas vehicles (buses, refuse collection vehicles, delivery trucks, etc.), and has the considerable advantage of being a storable, non-intermittent energy source. Producers can sell their biomethane production directly under the subsidized injection tariff scheme or – more recently – under a private contract directly with an industrial company (via an aggregator) in the form of a Biomethane Purchase Agreement (BPA), a carbon copy of the electricity PPA, adapted to the specific characteristics of biomethane.
To avoid the costs associated with purification, some producers valorize raw biogas production directly, without purifying it to transform it into injectable biomethane. Biogas can be used to produce heat and electricity through cogeneration (the engines used for cogeneration are often compatible with untreated or only slightly purified biogas), or directly in a boiler or industrial furnace that is not sensitive to gas quality. In this case, biogas is produced on the site where it is used. Depending on the country and region, raw biogas is either converted into electricity and heat (as in Germany and the United States), or transformed into biomethane for injection into networks (as in France), as shown in the figure below:
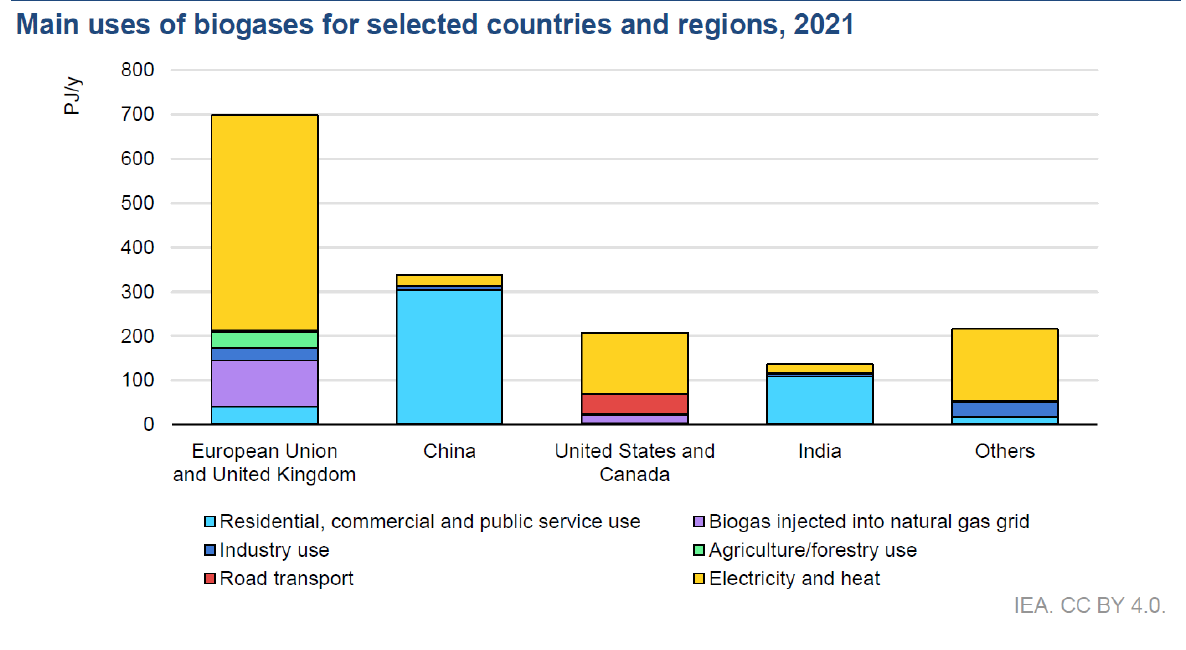
Combined global biogas and biomethane production reached over 1.6 EJ in 2022, an increase of 17% in 2017. Almost half of production is based in Europe, with Germany alone covering almost 20% of global consumption. A further 21% is produced in China, followed by the USA (12%) and India (9%).
Although the production costs of biomethane are generally higher than those of natural gas, biomethane is not subject to the price volatility from which natural gas suffers and, during the energy crisis, its price was lower in Europe and Asia (figure below):
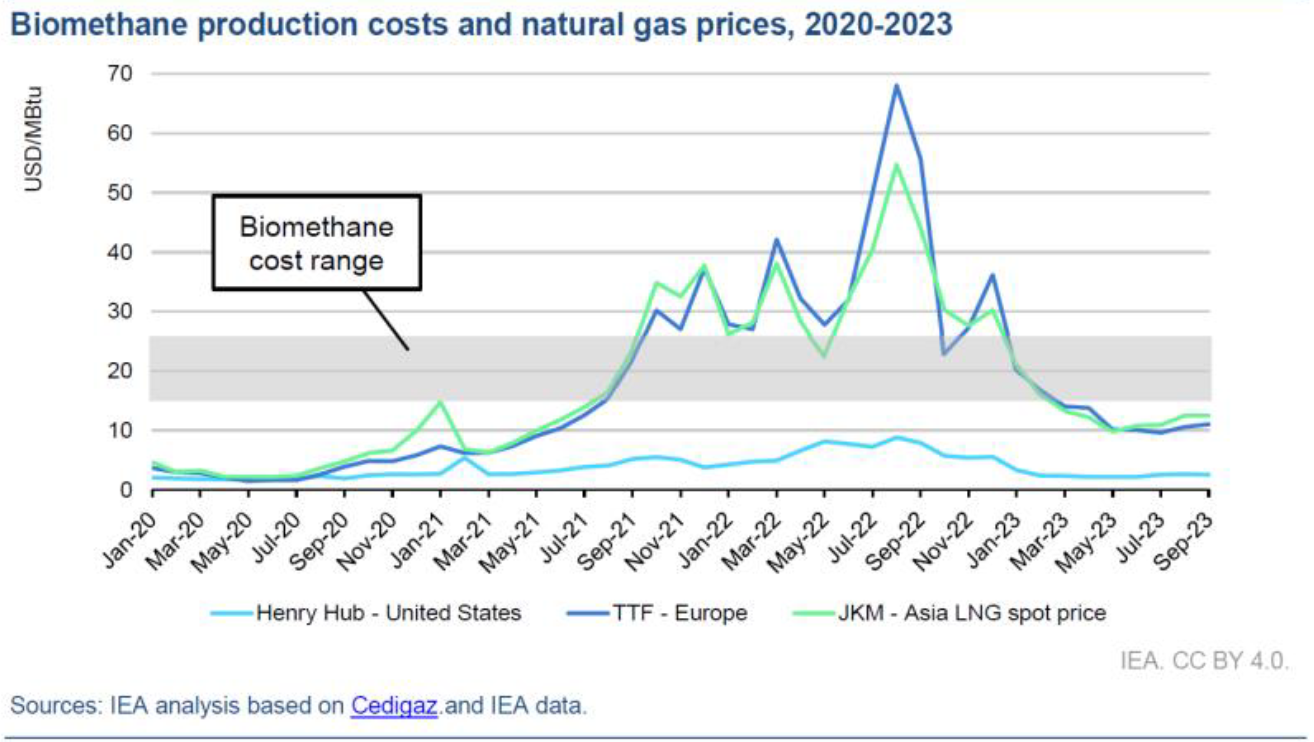
Compared with the 2017-2022 period, the International Energy Agency (IEA) forecasts accelerating growth in this energy, from 19% in 2017-2022 to 32% in 2023-2028, thanks to the adoption of proactive new policies in more than 13 countries in 2022-2023. The most significant growth will occur in Europe and North America, thanks to established infrastructure and experience and to past policies that make rapid deployment possible over a five-year period.
The IEA sees many advantages in this sector: it is a non-intermittent green energy, available wherever biomass is available, whose production cost is independent of spot gas and electricity prices; it also contributes to waste treatment and brings in additional income for the agricultural sector.
2.2. Other technologies to produce biogas or biomethane
Other technologies enable or will enable biogas or biomethane to be produced from other types of input, thus contributing to the increase in the production of these renewable gases called for by the IEA. These include landfill gas recovery, pyrogasification of dry biomass and hydrothermal gasification of sewage sludge.
Regarding landfill gas recovery, Waga Energy has been deploying a patented purification technology for several years now, marketed under the name WAGABOX®. This technology, which combines membrane filtration and cryogenic distillation, produces biomethane that is compatible with injection into the natural gas distribution network, whatever the concentrations of nitrogen and oxygen initially present in the raw gas. Some twenty projects of this type are currently in operation in Europe and the United States.
Dry biomass pyrogasification technology can be used to recover energy from other types of biomass: wood waste (forestry chips, waste from the furniture or paper industries, etc.) or Solid Recovered Fuels (SRF). Therefore, it does not compete with conventional methanization. While the production of low-calorific biogas by pyrogasification is a rustic technology that has already been commercialized for heat decarbonation applications on industrial sites, the production by pyrogasification of biomethane that can be injected into networks is much less mature. Indeed, to generate biogas of the purity required for injection, the pyrogasification process must take place in the complete absence of air, which greatly complicates the manufacturing process. There are a small number of projects of this type in the world, all at the pilot or demonstrator stage. ENGIE has developed its own technology in this field, which will be used in the Salamandre project, the first large-scale biomethane production facility (around 20 MW) to supply biomethane to the shipping company CMA-CGM. Commissioning is scheduled for 2027.
Finally, hydrothermal sludge gasification is an emerging technology still at the R&D stage. It will enable very dilute industrial or sewage plant effluents (sludge) to be transformed into biomethane, with a high level of efficiency (over 75%), thanks to a high-temperature, high-pressure thermochemical process. Since 2020, a pilot unit has been installed at the Paul Scherrer Institute (PSI) in Villigen, Switzerland.
3. Geothermal energy: why use electricity or gas to generate heat when hot water is naturally available underground?
Geothermal energy is a sustainable, cost-effective and weather-independent source of renewable energy. According to IRENA (International Renewable Energy Agency), geothermal energy can help stabilize electricity grids, partly offsetting the risks associated with the rapid deployment of variable renewable energies (wind and solar). Depending on the depth of the geothermal source and its characteristics, it can be used to produce heat, cold or renewable electricity. Surface geothermal energy (less than 200 meters deep) can be used to produce heat using a heat pump, as well as cooling. At these depths, it is not the high temperature of the subsoil that is exploited, but rather its stability over the seasons (warmer than the ambient air in winter, cooler in summer). Combined with a heat pump, it recovers the intermediate-temperature energy stored in the subsoil, thus “freeing up” part of the energy needed to heat or cool a building’s air-conditioning system. As a result, the coefficient of performance of heat pumps for heating or cooling buildings is significantly higher than that of heat pumps that simply draw their free energy from the ambient air. Surface geothermal energy can be installed anywhere, with the exception of areas where the subsoil is too congested.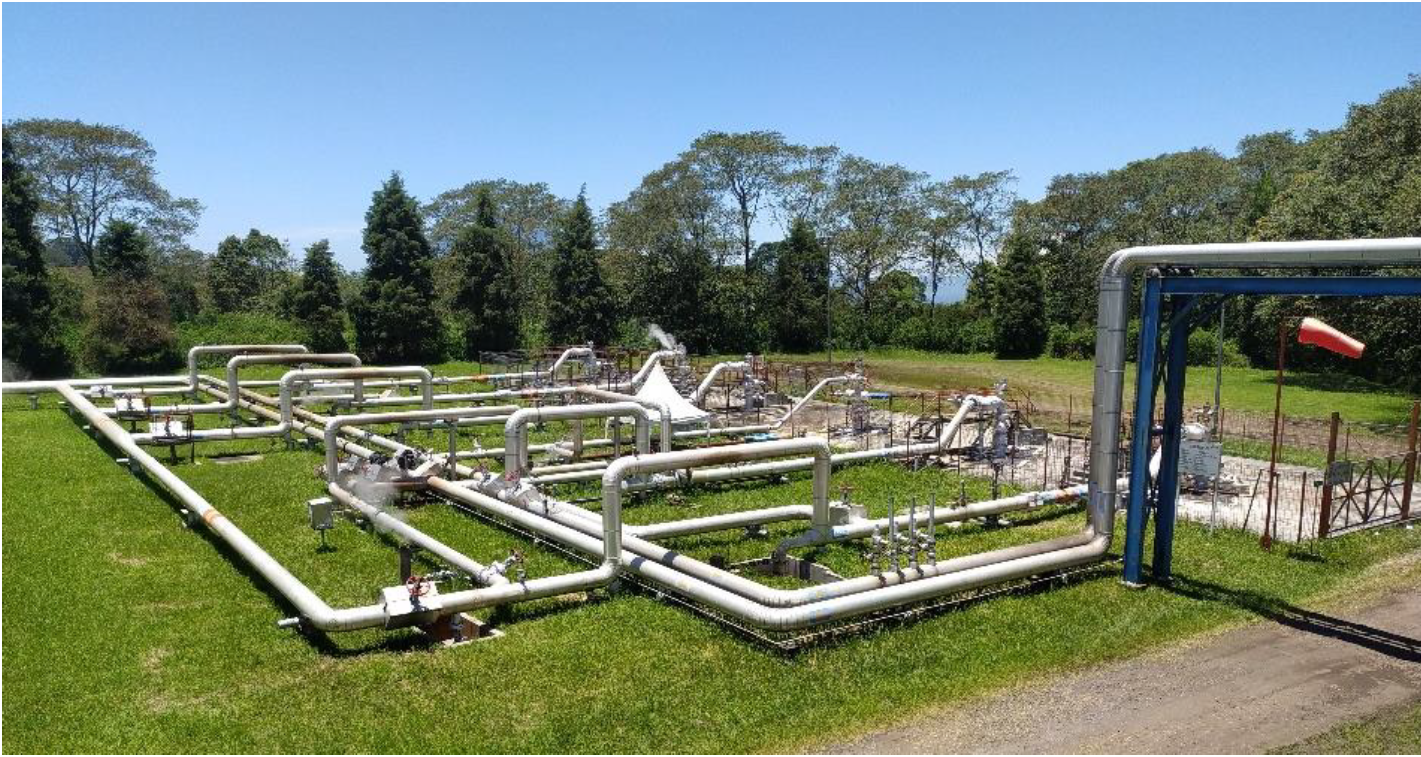
With deep geothermal energy (between 201 and 2000 meters deep), hot water or steam at between 90 and 150°C is extracted from deep underground. Depending on the temperature of the geothermal source, this energy can be used for district heating or to produce electricity and heat (cogeneration). These deep geothermal sources can be found in a large part of the Ile de France region. For example, the new geothermal power plant in Saint-Denis (93), inaugurated in December 2023 by the French Minister for Energy Transition, Agnès Pannier-Runacher, will supply the Olympic Village, then heat the equivalent of 7,500 homes.
Geothermal energy’s main challenges are the length of project development timescales, the importance of initial capital expenditure and the high risks in the early exploration phases. These challenges are related to difficulties in accessing financing, the complexity of fragmented regulatory frameworks, lengthy authorization procedures and a lack of qualified personnel. Another challenge is public acceptance, mainly due to a lack of information about geothermal technology and concerns about land use and environmental and social impacts.
Despite these – not insurmountable – obstacles, and given the renewed interest in renewable heat and switchable renewable energy sources in the wake of the energy crisis, the EU has increased its overall geothermal target, calling in May 2022 for a tripling of geothermal’s share of total EU energy demand by 2030.
Northern Germany, the Netherlands and Belgium in particular are key potential sites for geothermal energy supply. In France, applications for deep geothermal research permits are set to double by 2023, bringing to six the number of these projects, which have a high initial cost but are cost-effective in reducing greenhouse gas emissions. The French government is encouraging this movement through its stimulus plan (the “national geothermal action plan”, launched in February 2023).
4. Recovering waste heat… or second-hand heat!
As mentioned above, biogas and geothermal energy are two good ways of covering part of our thermal energy needs without using electricity grids. But there is another way: wherever possible, we can use the surplus heat available from those who release it into the environment. This is known as waste heat recovery.
Some uses of energy require equipment to be cooled, usually by cold production machines combined with passive devices that reject excess heat into the atmosphere or rivers. The principle of waste heat recovery is to capture this excess heat and make it available to another user, whether internal or external to the company producing it, to cover other energy needs.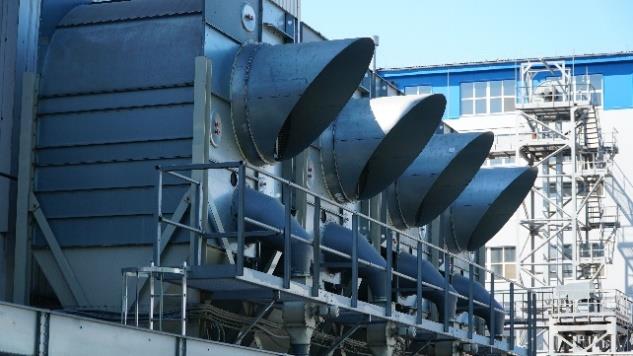
Computer centers are a case in point. These are very large consumers of electricity, and all the energy they consume ends up as heat that has to be evacuated. Heat can easily be recovered using conventional heat exchangers and injected into a district heating network, for example. So, instead of having, on the one hand, air coolers and refrigeration machines evacuating the heat from the computing center, and on the other, gas boilers delivering the heat to heat a district, the latter function is performed directly by the waste heat recovered from the computing center. The energy used to power the computers is therefore ultimately used twice!
Numerous projects of this type are already up and running all over the world. They are set to expand as energy prices rise.
This type of solution can also be used in industry, where various systems enable heat to be recovered at varying temperatures for a second use on the industrial site itself, or to cover a thermal need on a neighboring industrial site. For example, industrial laundries can quite simply recover heat from water at the end of the washing process to preheat pre-wash water, thereby saving 30% in consumption. Similarly, in the metallurgy and glass sectors, the recovery of high-temperature waste heat from industrial furnaces is commonplace.
It is important to stress that technologies for recovering waste heat at high or low temperatures for heating purposes are already mature and widely marketed (regenerative burners, heat exchangers, etc.). This is not so much the case when it comes to recovering low-temperature waste heat to power high-temperature industrial applications, which require highly specific heat pump technologies that are not always available on the market.
5. Heat storage: simpler and cheaper than electricity storage
Finally, it is worth remembering that heat can be stored very well, even over several months. When heat is produced with renewable electricity (e.g. with a heat pump), it can be very interesting to run the heat pump off-peak, and store the heat for use when needed. The same applies when you want to store waste heat produced continuously by an industrial process or computer center, for use in winter.
Three families of heat storage techniques exist, in order of increasing storage density (kWh/m3 ): temperature rise of a material (sensible heat storage), change of state of a material (latent heat storage of phase change); and chemical reaction between several compounds (thermochemical storage). For large-scale heat storage (heating networks, large tertiary buildings, industry), sensible heat storage is the simplest to implement. It often uses the subsoil, with numerous variants: buried probes, underground storage tanks, semi-underground tanks, aquifers, etc.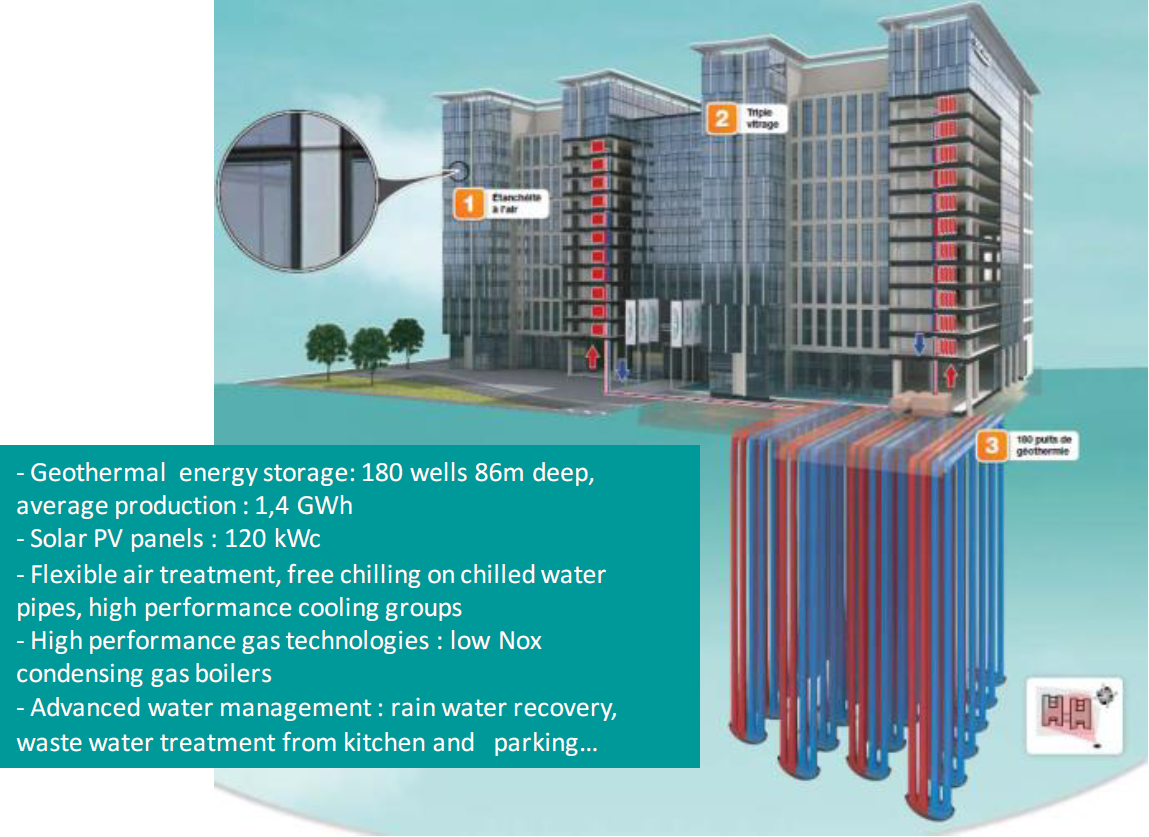
Buried probe technology is particularly well suited to new commercial buildings. For example, ENGIE chose this technology for its Brussels headquarters, which features 180 86m-deep wells under the building, capable of storing up to 1.4 GWh of heat or cold, in the form of hot or chilled water. This system, which is inexpensive to install when digging the foundations of a new building, allows the consumption of electricity to produce heat or cold to be completely decoupled from the period of consumption, while also optimizing the self-consumption of its PV panels.
These technologies can be applied in many different ways, with the advantages of being able to optimize the use of solar thermal or photovoltaic production, make the most of waste heat and avoid peak electricity consumption by heat pumps.
6. Summary and conclusion
Decarbonization through all-electric renewables is a formidable solution for decarbonizing part of the world’s energy mix, but it has its limits in terms of the need for permanent availability of switchable decarbonized energy and the difficulty of storing electricity. Even in countries that have chosen to develop or redevelop nuclear power, we will not be able to do without renewable gases or renewable heat, because short-term needs for decarbonized electricity are growing so fast that we risk creating bottlenecks such as the availability of raw materials (lithium, copper, cobalt, etc.), the inadequacy of production tools (battery gigafactories), or quite simply the inadequacy of non-infinite financing capacities for these new infrastructures.
From a risk mitigation perspective, it is reasonable to call for the diversification of decarbonization solutions, notably by developing parallel energy carriers such as biogas or biomethane, geothermal energy and renewable heat, combined with heat storage where necessary. Most of these technologies are mature, and can be implemented now, without waiting for other solutions to emerge in the medium or long term, such as low-carbon hydrogen, CO2 capture and sequestration (CCS) or new electricity storage technologies (solid batteries, liquid or compressed air storage, etc.).
The Energy Transition Management Team – Private Debt Expertise, Sienna Investment Managers
Bernard Blez, Senior Consultant – former R&D Director at Engie
Bibliography :
- “Batteries and Secure Energy Transition”, IEA report, 2024
- “Net Zero Roadmap – A Global Pathway to Keep the 1.5 °C Goal in Reach” – IEA publication 2023 Update
- “Clean Energy Market Monitor – IEA report – March 2024
- “Le stockage inter-saisonnier de chaleur – un atout pour le climat et la souveraineté” – Académies des Technologies – December 2023